The Earth
is certainly the most familiar planet, though it has only been a few
hundred years since we fully realized it was a planet. We begin our study
of objects in the Solar System with the Earth because it is interesting in
its own right, and it provides a test of many observing techniques that we
wish to use for other objects in the Solar System. |
The Earth is, at least by human standards, a beautiful planet, as the following images indicate.
 |
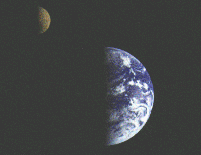 |
 |
Apollo 11 shot of Earth
(Ref)
|
Galileo shot of the Earth and Moon
| Galileo image of S. America
(Ref)
|
Here is an interactive viewer
that displays either a map of the Earth showing the day and night
regions at this moment, or a view of the Earth from the Sun, the Moon, the night
side of the Earth, above any location on the planet specified by latitude,
longitude and altitude, from a satellite in Earth orbit, or above various cities
around the globe (Credit:
John Walker).
Here is the NASA
Earth from Space
image gallery, where you can find many images of the Earth taken from space.
The Interior
The
study of the Earth's surface and interior is the domain of
geology.
We know little directly about the interior of the Earth. Most of our information
in that regard has come from seismic waves, which are vibrations in the body of the Earth.
Seismic Waves
There are two general categories of
seismic waves.
- P-waves, which are longitudinal pressure waves and can propagate in both solids and liquids.
- S-waves, which are transverse waves that can propagate in solids but not in liquids
Here is an
illustration
of the difference between P-waves and S-waves. These seismic waves, which are generated naturally by
earthquakes, by
volcanoes, and by
impacts,
and may be produced artificially by explosions and mechanical devices, tell us
about the interior in several general ways. The figure on the right illustrates
for a planet with varying interior density and a liquid core.
First, seismic waves have their direction of motion changed (refracted)
by variations in the interior density. Thus, by studying the way such waves
propagate in the Earth we can learn something about density variations. Second,
the fact that P-waves propagate in liquids but S-waves do not allows us to
determine if portions of the interior are liquid.
Structure of the Interior
Accumulated and detailed seismic studies, coupled with theoretical speculation, suggests the interior structure shown
schematically on the left (the figure is not to scale). The Earth is believed to
have a solid inner core, made mostly of iron and nickel. This is
surrounded by a liquid outer core, also mostly iron and nickel. The
diameter of the core is estimated to be 7000 km, compared with a 12,700 km
diameter for the entire planet. The crust is only a few tens of
kilometers thick. The region between the core and the crust is called the
mantle. The upper part of the mantle and the crust together are called
the lithosphere. Sitting just below the lithosphere is a region of
plastic consistency called the aesthenosphere. We shall have more to
say about the lithosphere and aesthenosphere shortly.
Geological Differentiation
The Earth did not have the interior structure described in the preceding
section when it was formed. The geological process by which the Earth came to
have its present interior structure is called differentiation, and is illustrated in the following figure.
 |
The process of geological differentiation
|
Within about 1 billion years of its formation the Earth was melted by heat arising from a combination of sources:
- Gravitational energy left from the formation of the planet,
- Meteor bombardment
- Decay of radioactive material trapped in the body of the Earth.
While the Earth was molten, gravity acted to concentrate more dense
material near the center and less dense material nearer the surface. When the
Earth solidified again (except for the liquid outer core) it was left with a
layered structure with more dense material like iron and nickel near the center
and less dense rocks nearer the surface. As the outer layers cooled and
solidified, large cracks developed because of thermal stress, leaving the
lithosphere broken up into large blocks or plates.
As we shall see, this has enormous implications for the subsequent geological
history of the Earth because it produces conditions favorable for plate
tectonics. One of the crucial questions that we will have of all solid
bodies in the Solar System is whether they have ever been differentiated.
The Atmosphere
The present atmosphere of the Earth is probably not its original atmosphere.
Our current atmosphere is what chemists would call an oxidizing
atmosphere, while the original atmosphere was what chemists would call a
reducing atmosphere. In particular, it probably did not contain oxygen.
Composition of the Atmosphere
The original atmosphere may have been similar to the composition of the solar nebula and close to the present
composition of the Gas Giant planets, though this depends on the details of how
the planets condensed from the solar nebula. That atmosphere was lost to space,
and replaced by compounds outgassed from the crust or (in some more recent
theories) much of the atmosphere may have come instead from the impacts of
comets
and other planetesimals rich in volatile materials.
The oxygen so characteristic of our atmosphere was almost all produced by plants
(cyanobacteria
or, more colloquially, blue-green algae). Thus, the present composition of the
atmosphere is 79% nitrogen, 20% oxygen, and 1% other gases.
Layers of the Atmosphere
The atmosphere of the Earth may be divided
into several distinct layers, as the following figure indicates.
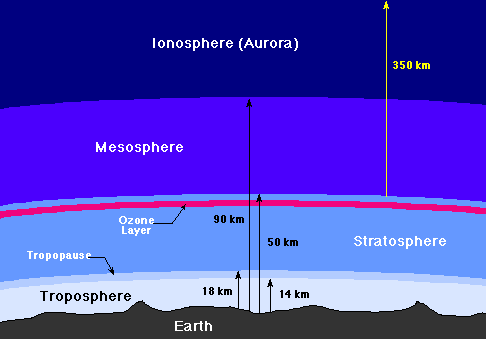 |
Layers of the Earth's atmosphere |
The Troposphere
The troposphere is where all weather takes
place; it is the region of rising and falling packets of air. The air pressure
at the top of the troposphere is only 10% of that at sea level (0.1
atmospheres). There is a thin buffer zone between the troposphere and the next layer called the tropopause.
The Stratosphere and Ozone Layer
Above the troposphere is the stratosphere, where air flow is mostly horizontal. The thin
ozone layer
in the upper stratosphere has a high concentration of ozone, a
particularly reactive form of oxygen. This layer is primarily responsible for
absorbing the ultraviolet radiation from the Sun. The formation of this layer is
a delicate matter, since only when oxygen is produced in the atmosphere can an
ozone layer form and prevent an intense flux of ultraviolet radiation from
reaching the surface, where it is quite hazardous to the evolution of life.
There is considerable recent concern that manmade flourocarbon compounds may be
depleting the ozone layer, with dire future consequences for life on the Earth.
The Mesosphere and Ionosphere
Above the stratosphere is the mesosphere and above that is the
ionosphere
(or thermosphere), where many atoms are ionized (have gained or lost
electrons so they have a net electrical charge). The ionosphere is very thin,
but it is where aurora take place, and is also responsible for absorbing the
most energetic photons from the Sun, and for reflecting radio waves, thereby
making long-distance radio communication possible.
The structure of the ionosphere is strongly influenced by the charged
particle wind from the Sun
(solar wind),
which is in turn governed by the level of Solar activity. One measure
of the structure of the ionosphere is the free electron density, which is an
indicator of the degree of ionization. Here are electron density
contour maps
of the ionosphere for months in 1957 to the present. Compare these
simulations of the variation by month of the ionosphere for the year
1990
(a period of high solar activity with many sunspots) and
1996
(a period of low solar activity with few sunspots):
Weather and Climate
Not only does the Earth have a complex atmosphere, but that atmosphere has
complicated motion and nontrivial behavior. We Earthlings call this
weather in the short term and climate over the longer term.
The following images illustrate some of the complex patterns that develop in Earth's atmosphere.
 |
 |
 |
Left: GOES-7 image of weather patterns in the Americas and Eastern Pacific. Middle: Hurricane Fran from GOES-8
(Ref). Right:
GOES-8 false-color image of water vapor in Earth's atmosphere
(Ref). |
For good measure, here are two galleries of hurricane images:
links to current
weather satellite images:
How does this
complex behavior arise? The most general answer is that it is a consequence of
(1) solar heating, and (2) effects associated with the rotation of the Earth. In
the next section we address these issues explicitly.
Consequences of Rotation for Weather
The Earth is a spinning globe where a point at the equator is travelling at
around 1100 km/hour, but a point at the poles is not moved by the rotation. This
fact means that projectiles moving across the Earth's surface are subject to
Coriolis forces that cause apparent deflection of the motion.
Coriolis Forces
The following diagram illustrates the effect of Coriolis
forces in the Northern and Southern hemispheres.
 |
The Coriolis force
deflects to the right in the Northern hemisphere and to the left in the
Southern hemisphere when viewed along the line of motion.
|
Solar Heating and Coriolis Forces
Since winds are just molecules of air, they are also subject to Coriolis forces.
Winds are basically driven by Solar heating. As the adjacent (highly idealized)
image indicates, Solar heating on the Earth has the effect of producing three
major convection zones in each hemisphere.
If solar heating were the only thing influencing the weather, we would then
expect the prevailing winds along the Earth's surface to either be from the
North or the South, depending on the latitude. However, the Coriolis force
deflects these wind flows to the right in the Northern hemisphere and to the
left in the Southern hemisphere. This produces the prevailing surface winds
illustrated in the adjacent figure.
For example, between 30 degrees and 60 degrees North latitude the solar
convection pattern would produce a prevailing surface wind from the South.
However, the Coriolis force deflects this flow to the right and the prevailing
winds at these latitudes are more from the West and Southwest. They are called
the prevailing Westerlies.
Cyclones & Anticyclones
The swirling motions evident in the
preceding animations are consequences of frontal systems anchored to high and
low pressure systems, which are also called anticyclones and
cyclones, respectively. The wind flow around high pressure
(anticyclonic) systems is clockwise in the Northern hemisphere and
counterclockwise in the Southern hemisphere. The corresponding flow around low
pressure (cyclonic) systems is counterclockwise in the Northern hemisphere and
clockwise in the Southern hemisphere. This is a consequence of the Coriolis
force, as illustrated for the Northern hemisphere in the following figure.
 |
Low pressure systems
(left) and high pressure systems (right) in the Northern hemisphere
|
Here is a pronounced example of a cyclone: a movie of
Hurricane Andrew
(653 kB). This animation is a loop of infrared satellite images
showing the path of Hurricane Andrew across Florida and into Louisiana from
Sunday, August 23 through Thursday, August 27, 1992 (Credit:
Nathan Gasser).
The Magnetic Field
The Earth has a substantial magnetic field, a fact of some historical
importance because of the role of the magnetic compass in exploration of the planet.
Structure of the Field
The field lines defining the structure
of the magnetic field are similar to those of a simple bar magnet, as illustrated in the following figure.
 |
The Earth's magnetic field and Van Allen radiation belts |
It is well known that the axis of the magnetic field is tipped with respect
to the rotation axis of the Earth. Thus, true north (defined by the direction to
the north rotational pole) does not coincide with magnetic north (defined by the
direction to the north magnetic pole) and compass directions must be corrected
by fixed amounts at given points on the surface of the Earth to yield true directions.
Van Allen Radiation Belts
A fundamental property of magnetic fields is that they exert forces on moving
electrical charges. Thus, a magnetic field can trap charged particles such as
electrons and protons as they are forced to execute a spiraling motion back and forth along the field lines.
As illustrated in the adjacent figure, the charged particles are reflected at
"mirror points" where the field lines come close together and the spirals
tighten. One of the first fruits of early space exploration was the discovery in
the late 1950s that the Earth is surrounded by two regions of particularly high
concentration of charged particles called the Van Allen radiation belts.
The inner and outer Van Allen belts are illustrated in the top figure.
The primary source of these charged particles is the
stream of particles emanating from the Sun that we call the solar wind.
As we shall see in a subsequent section, the charged particles
trapped in the Earth's magnetic field are responsible for the
aurora (Northern and Southern Lights).
Origin of the Magnetic Field
Magnetic fields are produced by the motion
of electrical charges. For example, the magnetic field of a bar magnet results
from the motion of negatively charged electrons in the magnet. The origin of the
Earth's magnetic field is not completely understood, but is thought to be
associated with electrical currents produced by the coupling of convective
effects and rotation in the spinning liquid metallic outer core of iron and
nickel. This mechanism is termed the dynamo effect.
Rocks that are formed from the molten state contain indicators of the
magnetic field at the time of their solidification. The study of such "magnetic
fossils" indicates that the Earth's magnetic field reverses itself every million
years or so (the north and south magnetic poles switch). This is but one detail
of the magnetic field that is not well understood.
The Earth's Magnetosphere
The solar wind mentioned above is a stream of ionized gases that blows outward from
the Sun at about 400 km/second and that varies in intensity with the amount of
surface activity on the Sun. The Earth's magnetic field shields it from much of
the solar wind. When the solar wind encounters Earth's magnetic field it is
deflected like water around the bow of a ship, as illustrated in the adjacent image
(Source).
The imaginary surface at which the solar wind is first deflected is called
the bow shock. The corresponding region of space sitting behind the bow
shock and surrounding the Earth is termed the
magnetosphere;
it represents a region of space dominated by the Earth's magnetic field in the
sense that it largely prevents the solar wind from entering. However, some high
energy charged particles from the solar wind leak into the magnetosphere and are
the source of the charged particles trapped in the Van Allen belts.
|